The group is working on the following topics:
- Device physics for quantum information processing.
- Mesoscopic superconductivity in new materials.
- Strongly interacting many-body systems.
- Applications of Machine Learning to characterize quantum devices.
Device Physics for Quantum Information processing. This area is the main focus of our current research effort. The majority of our recent publications related to topics related to developing quantum gates on superconducting qubits and techniques for qubit readout. We worked on two types of qubits: the transmon, the most common qubit, and the fluxonium, which offers certain advantages for quantum information processing: long coherence time, strong anharmonicity, and high-fidelity gates. For transmons, we are collaborating with Robert McDermott’s lab at our department. Based on the original proposal [58] of the SFQ control of the transmon, we demonstrated experimental implementation of this approach to qubit control [78], as well as we improved the strategy to generate pulse sequences to provide robust high-fidelity gates [71,80]. We also worked on the development [60,65,83] and demonstration [77] of a novel approach for qubit readout based on Josephson junctions’ interaction with quantized microwave fields. For the fluxoniums project, we developed and experimentally realized several approaches to realize two-qubit gates between fluxoniums [72,86,87,89,91]. We plan to continue improving qubit control to obtain high-fidelity gates for multiqubit systems, improve qubit readout, and develop techniques for the realization of quantum error corrections in superconducting quantum hardware.
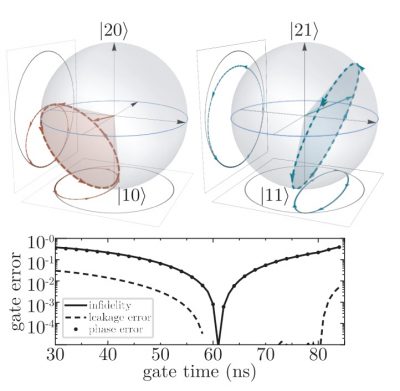
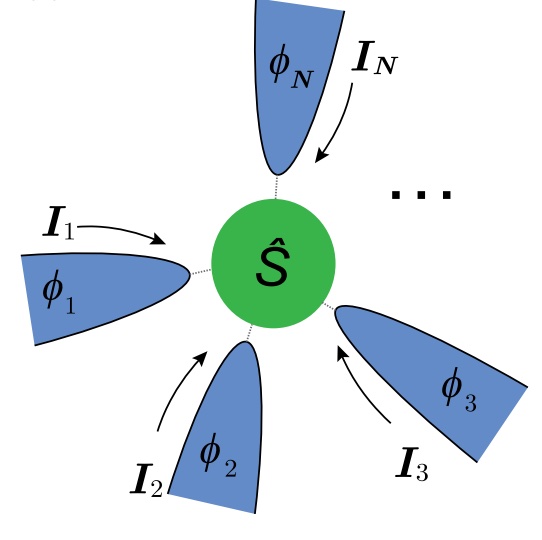
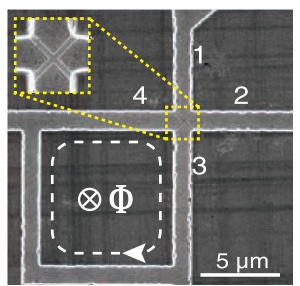
Mesoscopic Superconductivity. Most of the previous development of superconducting technology was based on two-terminal Josephson junctions. Recently, multiterminal junctions were fabricated. In these devices, a conducting layer connects three or four superconducting terminals on top of the epitaxially grown heterostructure. In this case, the current that flows through a specific terminal depends on superconducting phases and currents of all terminals. In paper [85], we reported a realization of such multiterminal devices in epitaxially grown superconductor-semiconductor nanostructures. We observe nontrivial geometric responses of the CCCs to the externally applied magnetic field and gate voltage, an inherent transport manifestation of multiterminal superconducting phase coherence. We collaborated on this project with experimental groups and the University of Maryland and NYU.
The multiterminal Josephson contacts were analyzed in purely theoretical papers with Alex Levchenko and Hong-Yi Xie [69,70]. Our other publications are related to mesoscopic superconductivity. One paper analyzes the regime of the Majorana fermion in a semiconductor nanowire, when the wire is placed on top of a superconductor with the magnetic field produced by nanomagnets [81]. Another paper analyzes the critical current distribution for the super-semi chaotic quantum dots [76]. We also proposed devices for quantum information processing as described in [74]. Our next steps are focused on microscopic simulations of superconductivity in super-semi materials using more powerful computational resources, such as those available at the UW Center for high-throughput computing – CHTC. The goal of these simulations is to characterize better microscopic parameters of materials. This work also helps in designing devices for quantum information processing and emulation of exotic states of matter.
Strongly interacting many-body systems. Strongly interacting Rydberg atoms were used to reveal surprising persistent oscillations of local observables. These oscillations have been attributed to a unique set of non-ergodic states called quantum many-body scars. Another important application of the interacting Rydberg atoms is to realize a spin-squeezed state to improve accuracy for precise time measurements. We collaborated with other groups at UW-Madison and at the Argonne National Laboratory to perform simulations to understand the effects of disorder on the dynamics of these Rydberg systems. We evaluated the threshold for the strength of disorder to significantly modify the system dynamics, such as suppressing the scar oscillations or diminishing the degree of spin-squeezing. The results were published in [84,88]. The next steps in this area are to analyze the effects of various forms of disorder on quantum dynamics and develop techniques to protect the system from the disorder by adequately engineering the interaction between the atoms and helping experimentalists interpret future experiments. Similar physics also can be explored in other systems. For example, we analyzed how a chain of interacting fluxonium qubits can study the transverse field Ising model [90]. This system was recently realized at the University of Maryland, and we are working to provide theory support for these experiments.
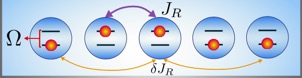
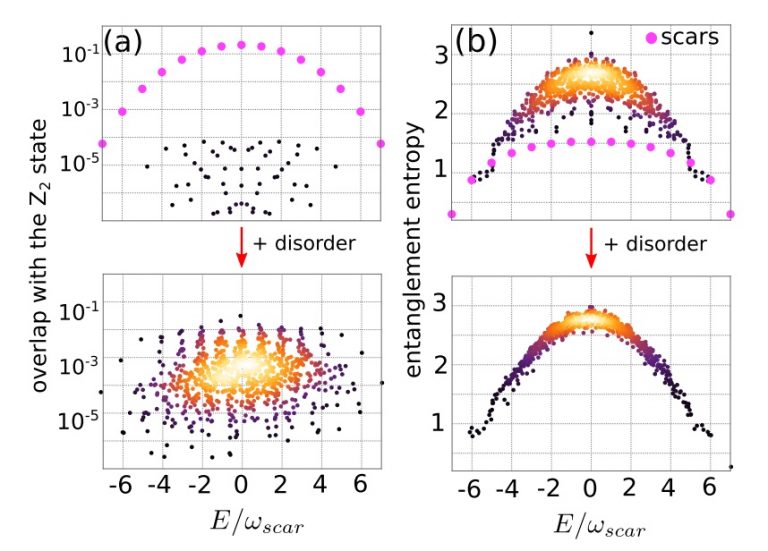